An illustration showing how electrons, which can have spin up or spin down, can form a stripe pattern in the Hubbard model. Recent groundbreaking calculations with this model are helping scientists better understand a class of high-temperature superconductors called cuprates. Credit: Lucy Reading-Ikkanda/Simons Foundation
Superfast levitating trains, long-distance lossless power transmission, faster nuclear magnetic resonance machines—all these fantastic technological advances could be within our grasp if we could just make a material that transmits electricity without resistance—or “superconducting” – at about room temperature.
In an article published in Science, the researchers report a breakthrough in our understanding of the origin of superconductivity at relatively high (though still cool) temperatures. The findings concern a class of superconductors that have puzzled scientists since 1986, called “cuprates.”
“There was great excitement when cuprate superconductors were discovered [in 1986]but without understanding why they remain superconducting at such high temperatures,” says Shiwei Zhang, senior research fellow at the Flatiron Institute’s Center for Computational Quantum Physics (CCQ). “I think it’s surprising to everyone that almost 40 years later we they still don’t fully understand why they do what they do.”
In the new paper, Zhang and his colleagues successfully recreated the characteristics of cuprate superconductivity with a simple model called the two-dimensional Hubbard model, which treats the materials as if they were electrons moving around a quantum chessboard. The breakthrough comes just a few years after the same researchers demonstrated that the simplest version of this model could not perform such a feat. Such clear patterns can lead to a deeper understanding of physics, says study co-author Ulrich Schollweck, a professor at the University of Munich.
“The idea in physics is to make the model as simple as possible because it’s hard enough on its own,” says Schollweck. “So at first we studied the simplest version we could imagine.”
In the new study, the researchers added to Hubbard’s 2D model the ability of electrons to make diagonal jumps, like bishops in chess. With this setup and thousands of weekly supercomputer simulations, the researchers’ model captured superconductivity and several other key features of cuprates previously found in experiments. By showing that Hubbard’s modest model can describe cuprate superconductivity, the authors demonstrate its value as a platform for understanding why and how superconductivity arises.
For most of the last century, physicists thought they understood why some materials were superconducting. They believed that superconductivity only existed at extremely low temperatures below minus 243 degrees Celsius (about 30 degrees above absolute zero). Such low temperatures require expensive cooling systems that use liquid helium.
When cuprates were discovered in 1986, they shocked the world of science with superconductivity at much higher temperatures. By the mid-1990s, scientists had discovered cuprates that remained superconducting down to about minus 123 degrees Celsius (about 150 degrees above absolute zero). Such temperatures can be reached using relatively inexpensive liquid nitrogen.
You can think of cupra as a lasagna of layers of copper oxide alternating with layers of other ions. (The name “cuprate” comes from the Latin word for copper.) Superconductivity occurs when electricity flows without resistance through layers of copper oxide. The simplest version of Hubbard’s 2D model uses just two terms to represent each layer as a checkerboard where electrons can jump north, south, east, and west.
“When I started working on Hubbard’s model in the early days of high-temperature superconductivity, we thought that once we had a clean model simulated on a small ‘checkerboard,’ we would fully understand superconductivity,” said study co-author Stephen White, a professor at the University of California, Berkeley. in Irvine. “But as we developed the techniques, we discovered that Hubbard’s model was much more complex than we thought.”
Quantum mechanics creates this complexity: The layers are inhabited by electrons, each with spin up or spin down. Electrons can become entangled. This entanglement means that the electrons cannot be treated separately, even when they are far apart, making them incredibly difficult to simulate on a computer.
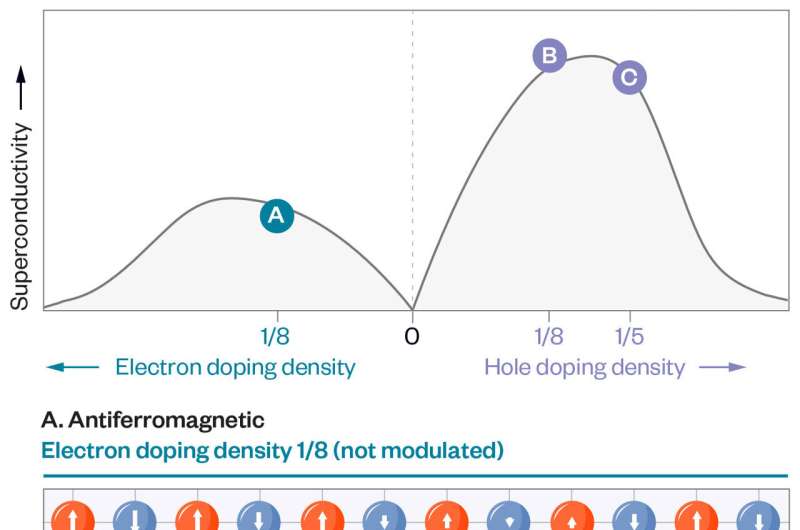
New research uses Hubbard’s two-dimensional model to investigate the emergence of superconductivity in a class of materials called cuprates. The model treats materials as electrons moving around a quantum checkerboard, with each electron having an up or down spin. When there are the same number of electrons as the checkerboard spaces, the system forms a checkerboard and is non-conductive. Adding electrons (in a process called electron doping) or removing them (in a process called hole doping after the vacancies left by the removed electrons) results in different levels of superconductivity (top panel). The bottom illustrations show electron or hole density along with spin patterns for three scenarios that indicate superconductivity. The first scenario (a) shows an antiferromagnetic pattern resembling a checkerboard of alternating up and down spins. The second (b) and third (c) scenarios show banding patterns of spin and hole density variations. Credit: Lucy Reading-Ikkanda/Simons Foundation
“Although Hubbard’s model can be written as an equation that takes only a line or two of text, because it applies to hundreds of atoms interacting through the strange laws of quantum mechanics, one can simulate it on a computer the size of the Earth in thousands of years old and we still can’t get the right answers,” says White.
Shortcuts are needed to deal with this level of complexity—and such shortcuts are the specialty of researchers. In the 1990s, White and Zhang separately developed now-famous techniques that exponentially reduce computer time. To deal with the highly complex pattern that comes from adding a diagonal jump, the researchers combined these two techniques. One technique views electrons more like particles; the other highlights their wavy structure.
“The great thing about the combination is that one is strong when the other is weak,” Sholvok says. “We can do a ‘handshake’ in a certain area where they both work, certifying one method using the other, and then explore the unknown where only one of them works.” Such a collaborative multi-method approach is the legacy of Simons’ collaboration on the problem with many electrons, which includes many CCQ scientists, he says.
Besides the quantum mechanical rules of motion, the number of electrons on the checkerboard affects the physics of the model. Physicists have known for many years that when there are the same number of electrons as there are spaces on the board, the electrons form a stable checkerboard pattern of alternating spins up and down. This setup is not superconducting—in fact, it’s not conducting at all. Therefore, cuprates require a change in the number of electrons.
In Zhang and his colleagues’ earlier work with Hubbard’s simplest model, adding or removing electrons did not lead to superconductivity. Instead, the stable checkerboard became a patterned pattern, with stripes consisting of either lines with extra electrons or lines with holes left by removed electrons.
However, when the researchers added the diagonal jump factor to Hubbard’s model, the stripes became only partially filled and superconductivity emerged. Moreover, the result roughly coincides with the experimental results for the properties of cupra.
“Do the stripes strictly compete with superconductivity, or do they cause superconductivity, or is it somewhere in between?” White asks. “The current answer is something in the middle, which is more complicated than any of the other answers.”
Zhang says the paper proves the continued relevance of Hubbard’s model and “classical” computing—that is, developing techniques and algorithms that make better use of ordinary computers, rather than waiting for quantum computers.
“After more than 30 years of intensive community effort without many reliable answers, it is often argued that solving Hubbard’s model will have to wait for a quantum computer,” Zhang says. “This effort will not only promote research in high-temperature superconductivity, but we hope it will also stimulate more research using ‘classical’ calculations to explore the wonders of the quantum world.”
More info:
Hao Xu et al, Coexistence of superconductivity with partially filled bands in the Hubbard model, Science (2024). DOI: 10.1126/science.adh7691
Courtesy of the Simons Foundation
Quote: Quantum breakthrough sheds light on perplexing high-temperature superconductors (2024, May 9), Retrieved May 9, 2024, from https://phys.org/news/2024-05-quantum-breakthrough-perplexing-high-temperature. html
This document is subject to copyright. Except for any fair dealing for the purposes of private study or research, no part may be reproduced without written permission. The content is provided for informational purposes only.